Table of Contents:
Hardly anyone visits the desolate outpost of Coldfoot, one of Alaska’s few communities outside the Arctic Circle accessible by road. Its 34 residents live in rustic accommodations along the Dalton Highway. The town’s highlights include an inn, a café, a gas station and a basic airport with a gravel landing strip. All day long, 18-wheeler fuel trucks thunder by on supply runs between Fairbanks and the oil fields of Prudhoe Bay further north. Some will stop to eat and tank up at Coldfoot because the next human habitation is 234 miles away, a town grimly named Deadhorse.
They say Coldfoot got its name from the days of the 1900 Gold Rush when miners would come as far as this remote settlement before getting “cold feet” and turning back. It’s still a lonely place, but one unexpected visitor showed up recently inside an infected Swainson’s thrush (Catharus ustulatus): the avian malaria parasite, Plasmodium circumflexum.
In 2011, scientists tested 676 birds representing 32 resident and migratory bird species captured from three northern locations in Alaska: Anchorage (61°N), Fairbanks (64°N) and Coldfoot (67°N). In Anchorage and Fairbanks, they found 49 birds infected by Plasmodium parasites. In Anchorage, even resident birds and hatchlings of species such as the boreal chickadee (Poecile hudsonicus), the varied thrush (Zoothera naevia) and the fox sparrow (Passerella iliaca) were found infected. The parasite was also detected in black-capped chickadees (Poecile atricapillus) and a myrtle warbler (Dendroica coronata coronata) in Fairbanks, indicating that transmission had occurred locally.
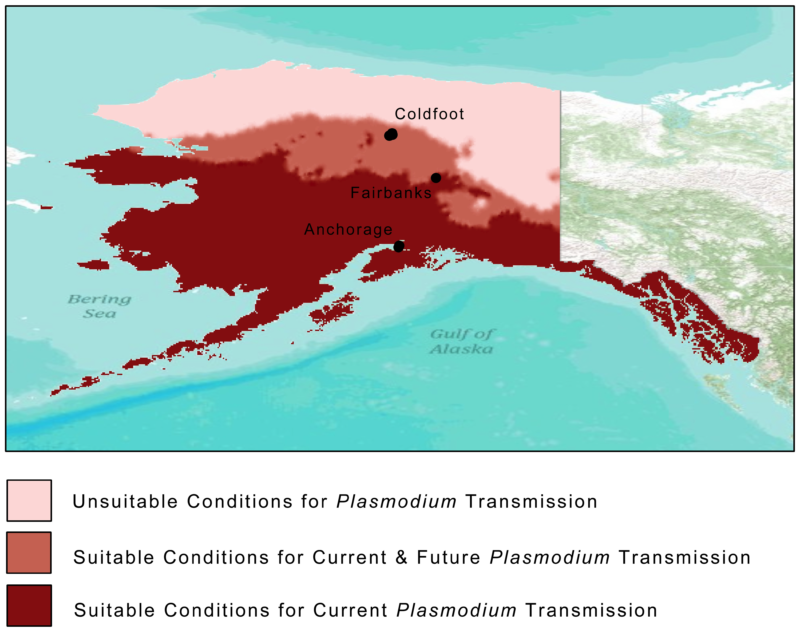
Climate change is changing Alaska, and mosquitoes are one of its beneficiaries. Growing seasons are getting longer and shrubs are coming up where once there were only grasses, thanks to disappearing sea ice, shrinking glaciers and terrestrial permafrost. With the Arctic region warming two to three times faster than the global average, scientists reckon it is only a matter of years before the parasite can complete its life cycle in places like Coldfoot, at the 67th parallel, and beyond. Plasmodium circumflexum is projected to enter the Arctic Circle by 2080, posing an existential threat to naïve (no prior exposure to malaria) species like snowy owls, whose immune systems have never been exposed to malaria.
The Arctic region is only one of several unprecedented locations where the avian malaria parasite has been detected in recent years. On a warming planet, avian malaria parasites are appearing in new geographies where climate change has made environmental conditions suitable for mosquitoes, including higher altitudes and once colder latitudes both northward and southward.
In Hawai’i, where invasive birds introduced the parasite in the early 1900s, it has wreaked havoc in recent decades and contributed to the extinction of several species. Since 2010, enabled by introduced host species, vectors and warming environments caused by climate change, the parasite has also debuted in New Zealand, the Galápagos Islands and the southernmost tip of Chile, in addition to Alaska.
Though research in this area is still developing, this could be the beginning of a boom time for the avian malaria parasite. Hitching a ride with host migratory birds, the emboldened parasites may find it easier to expand their presence into new areas that have never seen mosquitoes. Awaiting them there are many naïve and vulnerable bird species that have no experience or defenses against malaria such as the Siberian tit (Poecile cinctus) in Finland and the house sparrow (Passer domesticus) in Norway.
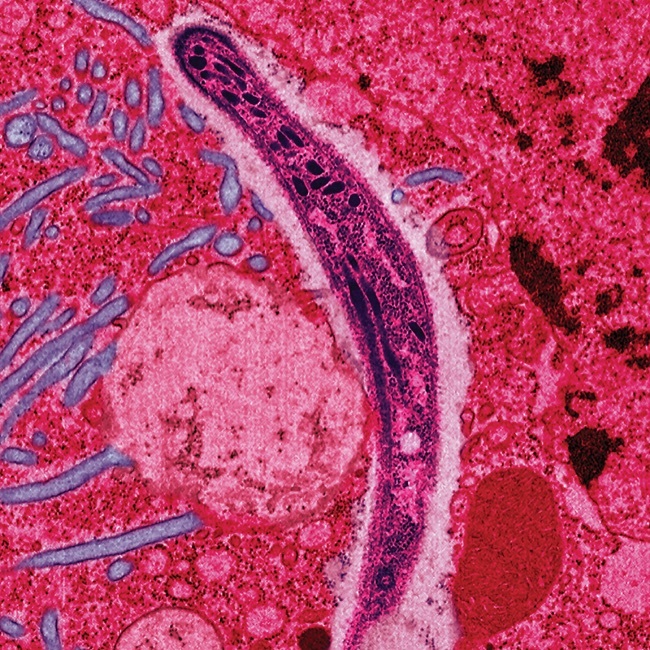
One parasite, different hosts
Why distinguish between avian and human malaria? After all, both are caused by single-celled protozoan parasites of the genus Plasmodium (even though the species vary). Both are transmitted through the bite of infected, pregnant female mosquitoes of different genera.
Human malaria, carried by the Anopheles mosquito, is caused by five species of the parasite of the genus Plasmodium, including falciparum, the most common, and vivax, knowlesi, ovale and malariae. Most people consider malaria a uniquely human disease but to Plasmodium it does not matter if the host is a human being, a blackbird, a penguin, a monkey, a snake, a zebra or some other vertebrate. Birds have been around much longer than humans, and the avian malaria parasite has co-evolved with them over millions of years. More than 50 parasite species of the genus Plasmodium have been linked with the transmission of avian malaria, with Plasmodium relictum being one of the commonest. The parasites are carried by Culicidae mosquitoes of the genera Anopheles, Culex, Aedes, Culiseta and Coquillettidia, and can complete their life cycle inside over 400 bird species covering 11 orders, including ducks, falcons, pigeons, and penguins. Perching birds (order Passeriformes), which include the songbirds, appear to be the most susceptible.
For millennia, all that humans knew about malaria was its symptoms. It was widely believed that the disease was caused by a ‘miasma’ or ‘bad air’ rising from swampy areas. Indeed, the disease derives its name from the Italian words for ‘bad air’ or mala aria. No one had made the connection with mosquitoes or even that birds, animals and humans could suffer from the same disease. Then, in two exciting decades starting from 1880, malaria’s secrets came tumbling out like pieces of a puzzle, with different pieces being discovered in England, Italy, Russia, and India.
Solving the malaria puzzle
Nearly everything we know about malaria grows out of the fact that it is caused by a coccidian, or a parasite that can only live and grow within the cells of a host animal. They also need a vector, such as a mosquito, to help them enter the host’s body.
It’s an ancient parasite. It has been found in the blood of mosquitoes preserved in amber 30 million years old. Malaria has been around for as long as Plasmodiums and vertebrates have existed together on Earth. References to what was almost certainly malaria exist in Chinese texts as old as 2,700 BCE, Mesopotamian clay tablets from 2,000 BCE, Egyptian papyri from 1,570 BCE and Hindu texts from 600 BCE. Greeks like Homer and Hippocrates described a disease of people living near marshy grounds characterized by high fevers, shaking, weakness and enlarged spleens.
There were many theories about how Egypt’s most famous pharaoh, Tutankhamun, died 3,300 years ago, but the most compelling evidence came from an insect. A genetic study of the pharaoh’s blood in 2010 revealed the DNA of Plasmodium falciparum, the most prevalent parasite causing human malaria. Tutankhamun’s death by malaria is generally accepted today and stands as the oldest verified case of malaria in the world.
A curious mind might wonder why a disease millions of years old was only fully understood barely a century and a half ago. The answer is that Plasmodium is a deceptive shapeshifter that morphs several times during its complex life cycle, changing its characteristics as it progresses through multiple stages in the host’s and the vector’s bodies.
Putting together a picture of this lethal and subversive journey was like assembling a jigsaw puzzle. Different scientists at different times stumbled upon one or another part of the mosquito’s life cycle, and it was not till 1898 that all the pieces came together to show that malaria, both human and avian, was a result of infection by the malaria parasite transmitted through the bite of a pregnant female mosquito.
The first clue appeared early one morning in an Algerian hospital nearly one-and-a-half centuries ago. Ironically, this was also the only finding based on a human infection. The crucial discoveries of the next two decades came from the blood of birds infected with avian malaria.
Dr, Alphonse Laveran, a professor of military diseases and epidemics, was bent over his microscope in the military hospital at Constantine, Algeria. In the wee hours of November 6, 1880, with winter well on its way, the air must have been cold, but the professor’s attention was elsewhere. He was riveted by what he had spotted in the blood of a French soldier who had been feverish with malaria for 15 days already: elongated cells of a kind he had never seen before, shaped like crescents and sausages. They were highly motile, propelling themselves with whiplash movements of their thread-like flagellum, much like tadpoles.
Some of these vivacious cells were along the edges of black pigmented granules he had already observed in the blood of malaria patients; others were wiggling along by themselves. He did not know then that the ‘granules’ were trophozoites and schizonts, a stage in the malaria parasite’s life cycle. The pigmentation came from hemozoin, a by-product formed when the parasite digests hemoglobin in red blood cells.
But it was the energetic, motile cell that had Dr Laveran’s total interest. He observed them in 148 blood specimens from 192 malaria patients. We know now that he had been looking at gametocytes but back then, convinced that he had found the protozoan parasite that caused malaria, he named it Oscillaria malariae. He notified the French Academy of Medical Sciences with a report titled New Parasite Found in the Blood of Several Patients Suffering from Marsh Fever.
It took Laveran another four years to convince scientists, including Louis Pasteur, that a single-celled protozoan parasite caused malaria. In 1907, he won the Nobel Prize for this discovery.
It was an epoch-making time in microbiology. A year earlier, Pasteur had developed a vaccine against chicken anthrax, proving beyond dispute that germs caused infectious diseases. Pasteur’s discovery laid the miasma hypothesis of malaria to rest once and for all. Everyone knew that they were looking for a pathogen.
Laveran’s discovery gave wings to malaria research, and several crucial discoveries quickly followed. In the early 1880s, Italian scientists Ettore Marchiafava and Angelo Celli, peering through Carl Zeiss Zena’s newly invented oil-immersion microscope, established that the parasite multiplied inside red blood cells, causing them to rupture and flood the blood with more parasites, which then invaded and reproduced within other red blood cells. A few years later, their colleague Camillo Golgi showed that this propagation happened at regular intervals which coincided with the typical attacks of high fever.
Still, no one knew how the parasites had entered the blood in the first place.
Meanwhile, unknown to Laveran and other Western researchers, Vasily J. Danilewsky, a Russian neurophysiologist studying Ukrainian birds, had found parasites in various stages of growth in blood samples from owls, rollers, finches, thrushes, buzzards and shrikes. He called them ‘pseudovermiculi’ and, like Laveran, he was particularly struck by an energetic, motile organism that whipped about using its flagella, reminding him of spermatozoa. By 1885, he had identified the three most common genera of blood parasites in birds, Plasmodium, Haemoproteus and Leucocytozoon, of which the first two are known to cause malaria. Five years later, Danilewski predicted that avian malaria would illuminate “the nature of malaria parasites of man and general observations on malaria”.
Unfortunately for Danilewski, much of his research was published in Russian and it was not until 1889, when they were translated into French, that they got the world’s attention.
A big question remained unanswered: how did the malaria parasite find its way into the host, whether human or avian? In 1894, Sir Patrick Manson, recognized as the ‘Father of Tropical Medicine’, surmised that the mosquito was very likely to be the vector that carried the malaria parasites from person to person. He had demonstrated as far back as 1877 that the filarial worm, which causes elephantiasis, used the mosquito as an intermediary. But Manson lived in London, a city not famous for its mosquitoes, and it fell to another Englishman, Ronald Ross, to prove that Manson’s theory was correct.
A remarkably gifted British officer in the Indian Medical Service in Secunderabad, Ross had observed the whipping movements of crescent-shaped Plasmodium falciparum parasites in the blood of a dapple-winged Anopheles mosquito that had fed on a malaria patient just four days earlier. Two years later, he noticed dark pigmented cells growing in size on the mosquito’s gut walls. Alas, just at the edge of a momentous discovery, he was transferred to Calcutta, a city with little human malaria.
Unrelenting, he continued his research using larks and other birds instead of humans, and Culex mosquitoes instead of Anopheles. Finally, in July 1898, he demonstrated that mosquitoes were indeed the vectors for avian malaria. He found that malaria parasites thrived within mosquitoes, developing into sporozoites and migrating to the insects’ salivary glands. The next time it took a blood meal from a human, bird or other animal, the sporozoites would pass from the mosquito into the new host.
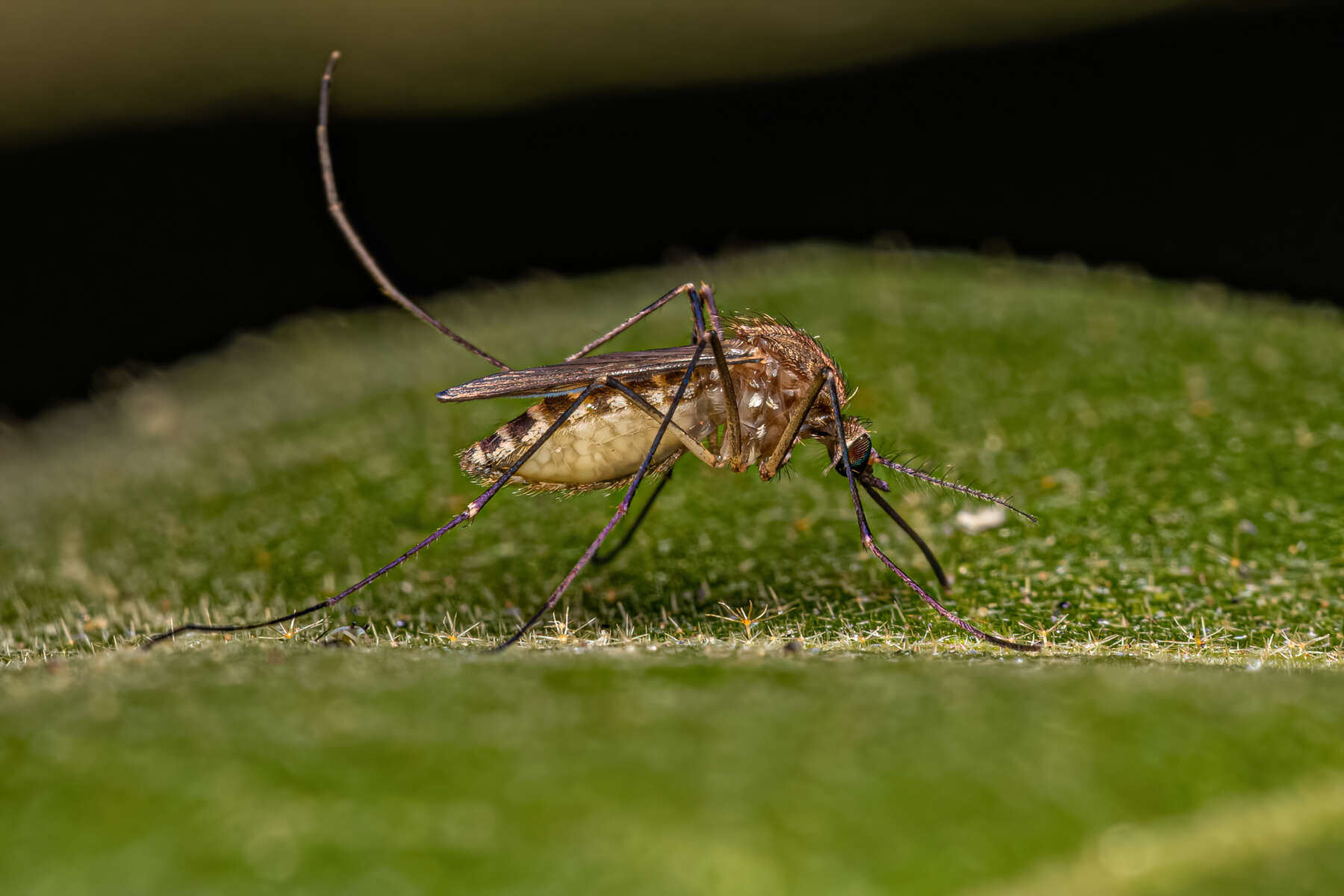
A parasite’s double life
The avian malaria parasite’s life cycle begins with sporozoites lurking in the saliva of a blood-feeing female mosquito, waiting for a bite to pass from the mosquito into the bird’s bloodstream or deep into its skin. Here, they develop into meronts within cells in the liver, spleen and bone cells all over the body. Within 36 to 48 hours, meronts progress to the next stage, rupturing to release merozoites into the blood. These, in turn, invade red blood cells, reproducing within them asexually until the cell bursts, releasing even more merozoites to infect and replicate within new blood cells. The classic high fevers and paroxysms at the acute stage of malaria infection happen when the red blood cells burst periodically.
Some merozoites develop into gametocytes and float in the blood, waiting to enter a mosquito as it feeds.
Within the mosquito, the parasite enters a sexual phase. The gametocytes develop into male and female gametes and unite to generate new sporozoites that migrate to the insect’s salivary glands. When the insect has its next blood meal, it will inject saliva into the feeding spot to prevent clotting. This is when the sporozoites will enter the host, starting the infection cycle all over again.
Not all infected birds sicken or die. Some wild birds adapt to tolerate a low, chronic level of infection without suffering severe health consequences and effectively become asymptomatic ‘reservoirs’ of the parasite. Studies have shown that the parasite causes deep damage at the genetic level even to reservoir species by shortening the length of their telomeres, which are like protective caps at both ends of a chromosome that shield the DNA from harm. Longer telomeres generally imply a longer life span. To make matters worse, malaria-infected, asymptomatic female birds will pass on their abbreviated telomeres to their healthy chicks, effectively creating new generations of birds with shorter life spans.
Avian malaria parasite species differ from each other in host range, geographic distribution, competent hosts, and pathogenicity. For example, one strain of parasite might cause mild disease in a specific bird species within a narrow range of environmental conditions. Another might be capable of surviving in a range of environments and cause severe disease or even death in several bird species. Being so versatile significantly increases the threat avian malaria poses to bird species as it spreads to new areas, sometimes facilitated by warming temperatures induced by climate change. Naïve birds such as penguins are especially at risk and suffer immensely, becoming anemic with weakness and appetite loss as the disease destroys their red blood cells. Some become comatose and die.
Avian malaria has been on the rise worldwide since the 1940s, particularly in Africa and Europe, major bird migration corridors. The disease has also become more common among certain species such as house sparrows (Passer domesticus), great tits (Parus major) and blackcaps (Sylvia atricapilla) and has been detected in the Galápagos penguin (S. mendiculus), a species once thought to lie beyond the geographical reach of Plasmodium. The factors underlying the spread of avian malaria remain unclear but habitat loss, introduced predators, disease and environmental changes have been implicated.
Mosquitoes rising
The world map is being redrawn at an unprecedented scale and speed by climate change. Some changes, such as droughts, might dry up landscapes and reduce mosquito populations but others will help them grow in abundance and diversity in emerging geographies with new and possibly naïve hosts. The experiences of Hawai‘i have already shown that altitude, rainfall and warming temperatures influence the spread and development of Plasmodium parasites, creating new ponds and stagnant pools for mosquito larvae to breed.
Rising sea levels will inundate entire landscapes and form new coastlines, creating new territories for mosquitoes to invade. Salt marsh mosquitoes, typically coastal, may start showing up in areas deeper inland. In wetlands, where climate change’s effects are more measurable, increased sea levels and marsh edge erosion from intense storms are already visible. Vegetated salt marshes are being converted into open tracts of standing water. Mosquitoes will not be far behind.
If we have one more warm year, we’re not going to have any birds left.
Dr. hanna mounce
As climate change accelerates, so will its consequences. Studies indicate that a warming planet will increase the transmission rates and patterns of mosquito-borne diseases and widen their geographic range. There is an urgent need to better understand how Plasmodium persists and what conditions trigger its growth.
As mosquitoes expand their domains to previously inhospitable locations now made amenable by climate change, they will meet new and naïve bird species like Hawaiian honeycreepers that are utterly vulnerable to avian malaria, and at extremely high risk of extinction from it. Climate change also creates opportunities for host-switching, whereby a mosquito develops a vector-host relationship with a bird species it has never encountered before. Early climate change studies indicate that new parasites can emerge and switch from one host to another even without any evolutionary changes, a process known as ecological fitting.
Three places on the planet are on the frontlines of rejuvenate avian malaria, each at a different stage of the invasion. In Hawai‘i, the disease is entrenched and still evolving, driving entire species to extinction and displaying the full scope of its lethality. In New Zealand, where warming weather is helping the parasite cross over into terrains once too frigid for it to breed, it directly threatens the endangered, yellow-eyed penguin, hoiho. A thousand miles west of Ecuador, in the Galápagos Islands, the parasite has not claimed any victims yet but has been detected in several birds, including the endangered Galápagos penguin.
Case Study 1: Hawai’i
Creeping Death on the Mountain
The precise start of Hawaii’s avian catastrophe can be traced to a moment in 1826 when some sailors recharging their ship’s water supply dumped stagnant water from barrels into a canal in Maui. Unknown to them, the water they jettisoned contained thousands of larvae of Culex quinquefasciatus, the night-flying southern house mosquito.
Reports of a new insect reached the Reverend William Richards, in charge of the Mission Station at Lahaina, Hawai‘i. According to a native Hawaiian he spoke to in 1827, this nalo (fly) had never been seen in these islands before and made its presence known by “a singing in the ear”. Complaints of an itch caused by this singing insect had been coming for some time, mainly from Hawaiians living near pools of standing water or streams in Lahaina.
The trail led back to the HMS Wellington, a 74-gun Navy ship that had traveled from Mexico to Lahaina and unwittingly introduced the malarial mosquito into a pristine Eden that had known neither mosquitoes nor malaria till then.
The avian malaria Plasmodium might already have existed in Hawaii’s islands for thousands of generations, brought by shorebirds and other waterfowl or via introduced non-native parasite-carrying passerine birds. However, in the absence of a mosquito vector the parasite could not be transmitted from bird to bird.
All that changed in 1826. Today, makika, as Hawaiians call mosquitoes, are found across the archipelago, infecting dogs with heartworm and birds with avian malaria and avian pox. By the end of the century, black-and-white striped ‘tiger mosquitoes’, Aedes aegypti and Aedes albopictus, had also arrived.
The Hawaiian Islands once supported 113 endemic bird species. They ranged from flightless geese, ibis and rails to the iconic and beloved Hawaiian honeycreepers, descended from a species of Eurasian rose finches that came here seven million years ago. More than 50 new species of honeycreepers evolved with astonishing speed, diversifying into a range of bill sizes and shapes in iridescent colors of yellow, green, orange, red and others. Honeycreepers are uniquely Hawaiian, cherished and woven into the culture and fabric of social life. Over time, they adapted to live in harmonious balance with the plant, animal and insect species with whom they shared the native forests.
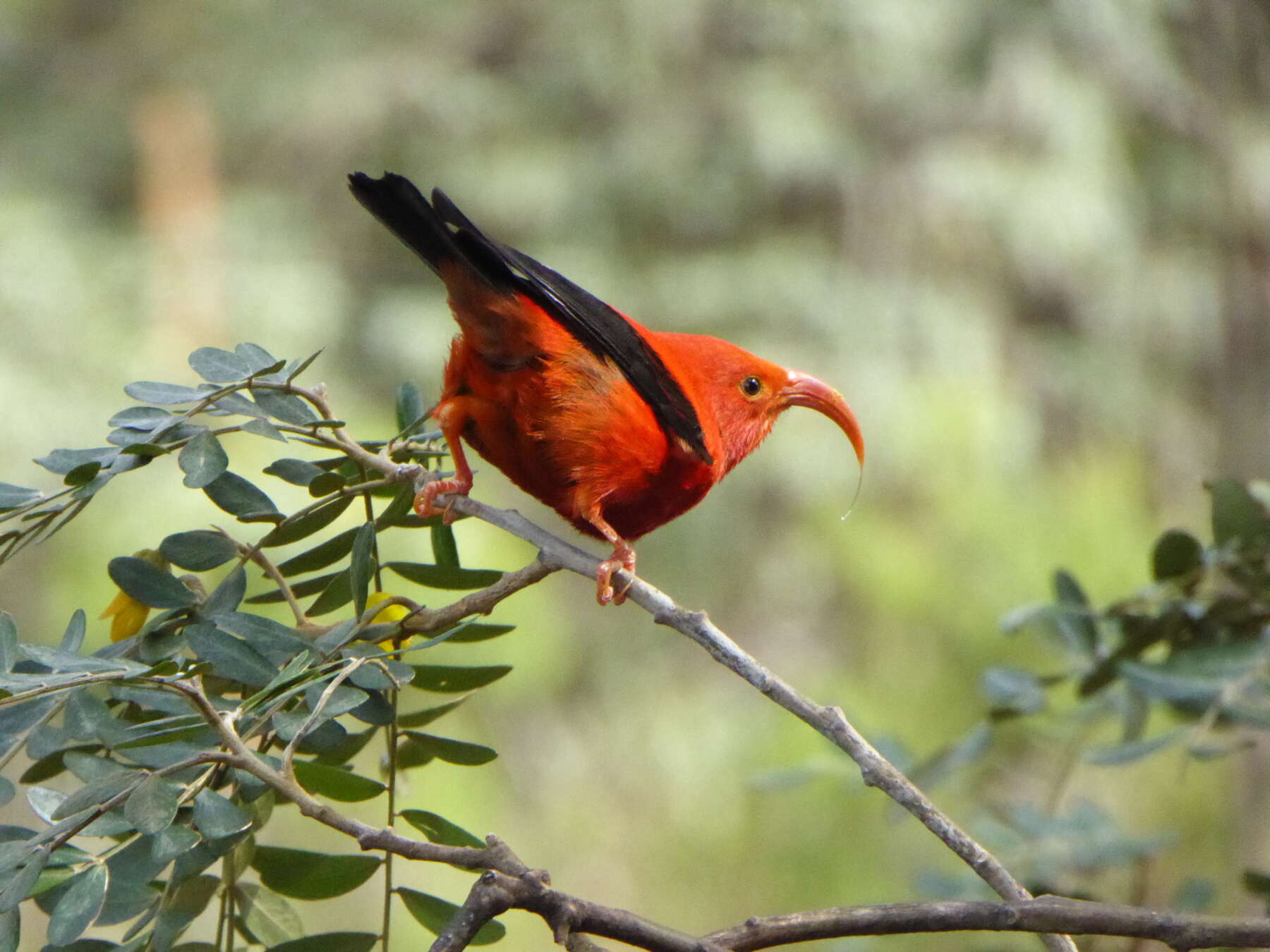
The first threats came with the first humans. Polynesians, the earliest settlers, cleared forest land to make room for farming and cultivation, depriving birds of their habitat and driving them inland. The arrival of Europeans and Western colonizers about 200 years ago marked the beginning of a spiraling ecological catastrophe driven by more habitat destruction and degradation, the introduction of invasive non-native plants and predators such as rats and dogs, and worst of all, new diseases such as avian pox and avian malaria.
The toll has been heavy: 71 bird species have been confirmed lost, 48 before the Europeans arrived, and 23 since Captain Cook set foot here in 1778. Of the 59 species of forest birds that co-existed with humans, 33 are now extinct or presumed to be. Of the remaining 26 species still around today, 24 are listed by the International Union for the Conservation of Nature (IUCN) as vulnerable, near-threatened, threatened, endangered, or critically endangered.
The single largest threat to honeycreepers today is avian malaria. Plasmodium relictum has driven all but 17 of the honeycreepers to extinction. In just 200 years, the parasite wiped out most of a family of birds that nature took 7 million years to create. Faced with disappearing habitats and growing threats from predators and diseases, the remaining birds migrated to higher elevations too cold for mosquitoes to breed. A 3-7 year study in 2015 looked at the prevalence, transmission and mortality of avian malaria across a range of elevations in forests on the island of Hawai‘i for the ‘apapane (Himatione sanguinea), Hawai‘i ‘amakihi (Chlorodrepanis virens) and ‘i‘iwi (the Scarlet Honeycreeper, Drepanis coccinea). The study found malaria infection was highest at low elevations, moderate at mid-elevations and limited in high-elevation forests.
In Hawaii, the threshold temperature below which Plasmodium relictum cannot develop to its infective stage is estimated to be 55.4°F, but Plasmodium is most prevalent in wild mosquitoes in mid-elevation forests where the mean summer temperature is a comfortable 62.6°F. As Plasmodium moved up the mountain, so did the birds. With their numbers under assault from avian malaria, they sought safe ground in forests above 5,000 feet, too cool for mosquitoes to thrive.
But Plasmodium has time and climate change on its side. Hawaii’s minimum night temperature, the primary determinant of the elevation mosquitoes will find tolerable, has been inching up steadily by 1°F per decade on average because of climate change. Soon, even the highest altitudes in Hawaii may no longer be Plasmodium-free. Over the last 60 years, mosquitoes have been advancing up the mountain, from 2000 feet in the late 1960s to nearly 5000 feet today. In Kauai, most of the remaining birds were refugees on a slender band of forest on the Alaka‘i Plateau. Today even that refuge is no longer safe.
“If we have one more warm year, we’re not going to have any birds left,” says Dr Hanna Mounce, Program Manager of the Maui Forest Bird Recovery Project (MFBRP). At particular risk are four species of honeycreeper – the ʻakekeʻe (Loxops caeruleirostris) and the ʻakikiki (Oreomsytis bairdi) on Kaua‘i island; and the kiwikiu (Pseudonestor xanthophrys) and the ʻākohekohe (Palmeria dolei) on Maui.
Three main initiatives being considered to save the endangered honeycreepers from extinction are landscape-level mosquito control through the Wolbachia incompatible insect technique (IIT); captive care; and conservation translocations. The Kauaʻi Forest Bird Recovery Project (KFBRP) is field-testing the use of a larvicide made from a naturally occurring soil bacterial strain called Bti (Bacillus thuringiensis israelensis). Historically, Bti products have been used for treating waterways worldwide for over 30 years, and are approved in the U.S. for use in organic agriculture. WHO has approved their use in drinking water.
In Maui, Birds Not Mosquitoes (BNM), a consortium of over a dozen state, federal, industry and conservation partners, including the MFBRP, is doing trials with IIT, which has so far only been tried on mosquitoes carrying human diseases. In China, the use of IIT slashed dengue-carrying mosquito populations by 90% on two islands.
IIT takes advantage of the fact that to produce offspring together, both mating mosquitoes must be carrying a particular strain of Wolbachia, a naturally occurring bacteria that lives in the insect’s gut. Beginning in November 2023, BNM plans to air-drop 250,000 male Culex mosquitoes over 3,000 acres of habitat critical for honeycreepers in East Maui. These insects, with a strain of Wolbachia different from the one prevalent in the wild female Culex mosquitoes in Maui, will be clustered within biodegradable pods the size of mangoes, each holding about 1,000 mosquitoes.
Case Study 2: New Zealand
From Singing Blackbirds to Dying Penguins
It is ironic that the first malarial parasites to enter New Zealand came in under the umbrella of an activity called ‘acclimatization’, driven entirely by a simple sentiment of the early European settlers: homesickness. Missing the familiar sights and sounds of home, they formed voluntary associations called acclimatization societies dedicated to importing mainly European birds, animals and plants to make the new land feel more like good old England. In an era before environmental sciences and ecological awareness, the hope was that the introduced species would gradually ‘acclimatize’ and adapt to their new locations. No one suspected that there would be an unexpected stowaway with the shipments.
The first blackbirds (Turdus merula) were brought in around 1861 by the Auckland Acclimatisation Society, along with song thrushes, starlings, skylarks, sparrows, goldfinches, magpies, doves and pigeons, and others. The settlers had missed the beloved bird’s sweet song, but they also hoped it might eat some of the caterpillars, moths and beetles that were damaging crops.
When avian malaria caused by the Plasmodium species showed up 60 years later, in the 1920s, it was in blackbirds and song thrushes (Turdus philomelos), which had by then spread everywhere and become a common sight in New Zealand. Over the last few decades, avian malaria has been flagged as a potential emerging disease, with at least 17 identified Plasmodium species, none endemic to New Zealand but all highly prevalent among introduced birds. Blackbirds, found to carry at least three Plasmodium strains, are believed to be the main reservoir for the parasite. Avian malaria has been detected in 35 different bird species. It has caused death in threatened species such as the dotterel (Charadrius obscurus), the South Island saddleback (Philesturnus carunculatus), mohua (Mohua ochrocephala), hihi (Notiomystis cincta) and two species of kiwi (Apteryx spp.).
The parasite is transmitted mainly by two mosquito species out of the 12 indigenous and four introduced species in New Zealand. One of them, Culex quinquefasciatus or the southern house mosquito, has already brought Hawai‘i to the brink of ecological disaster and driven dozens of bird species there to extinction. Another species, Culex pervigilans, seemed to be the vector in places not covered by C. quinquefasciatus.
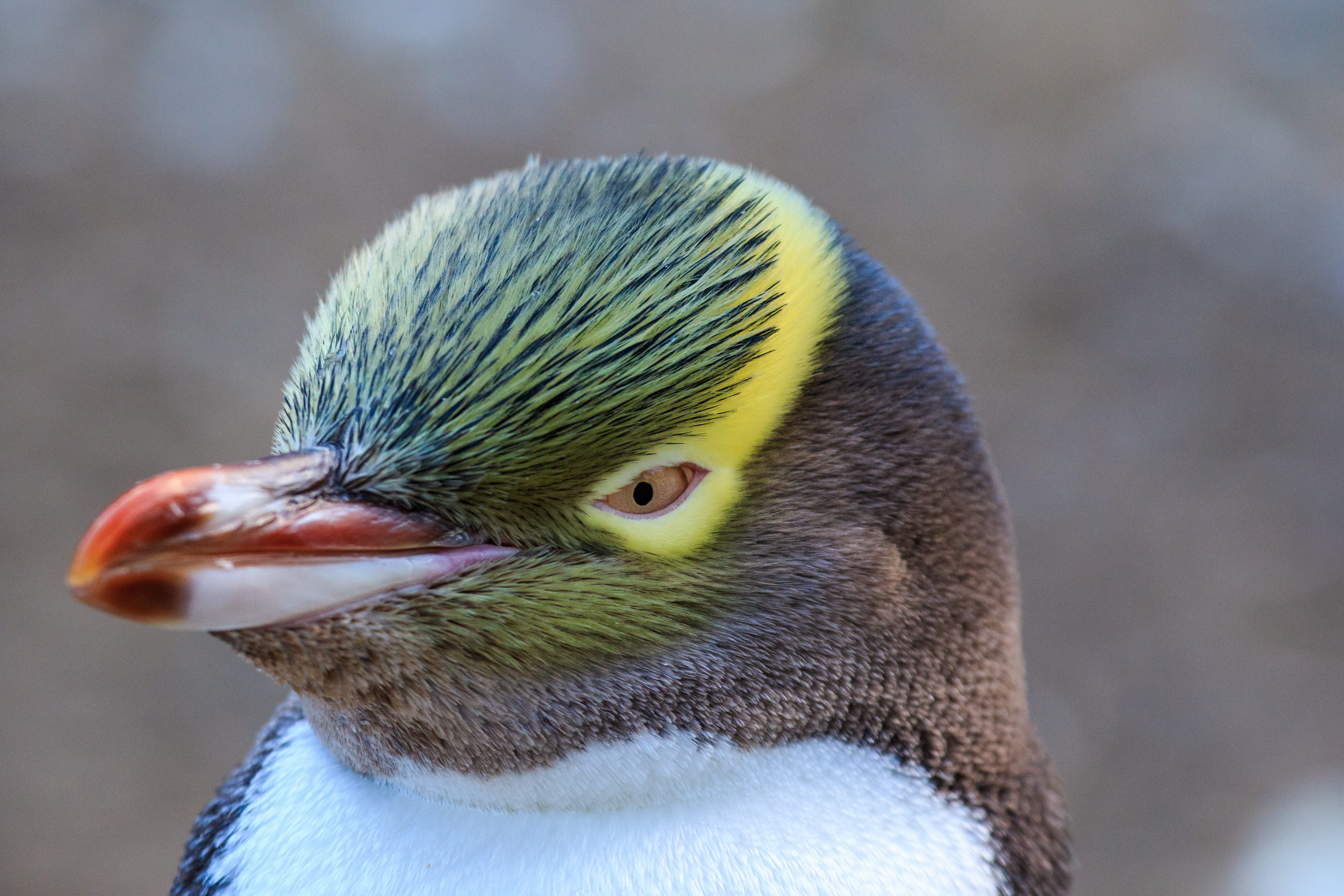
As in Hawai‘i, warming temperatures caused by climate change have enabled Plasmodium to creep southwards and enter once inhospitably cold areas such as Otago and Southland, the territory of the bird that adorns New Zealand’s 5$ note: the yellow-eyed penguin (Megadyptes antipodes), known locally as hoiho. A 2007 University of Queensland study estimated that penguin tourism generates about $100 million annually. But hoihos are an embattled species today, facing the threat of extinction. The number of breeding pairs in Otago and Southland, 600 in 2009, has dropped by almost 70% to 165 in 2020.
The causes? Dog attacks, loss of habitat, pollution, the stresses caused by too many tourists wanting selfies with the birds — and a disease that had not been detectable here before 2018, avian malaria. Hoiho are considered naïve; having evolved for millions of years without encountering the avian malaria parasite, they have no defenses against it.
Malaria expert Bruce Russell, associate professor at the University of Otago, has a dire prediction. “It’s a disaster,” he says. “They’re all going to be gone [from the mainland] in 10 years if we don’t do something.”
Russell and his team, working with the local Penguin Rescue Rehabilitation facility, have been saving 30 penguins a year for the last two years by treating them with child doses of Malarone, a human antimalarial drug.
Russell misses better drugs and diagnostics designed for penguins. “We want something that’s rapid, specific and sensitive. We want to be able to quickly identify penguins who are sick.” He believes that Tafenoquine, a human anti-malarial drug developed by the Australian Defence Force and the US Army, could work as a prophylactic for hoihos, protecting them from malaria for about a month during the warmer season.
Case Study 3: Galápagos Islands
A Looming Disaster in Eden
Most people think of penguins as birds that dwell in cold, icy regions and would be surprised to hear of a penguin that nests near the equator. Standing at just around 20 inches tall and weighing in at about 4.4 lbs, the Galápagos penguin (Spheniscus mendiculus) is the world’s smallest after the fairy penguins of New Zealand, one of the rarest and the only one living north of the equator and adapted to a tropical latitude. It has been on the IUCN’s Redlist of Endangered Species for 13 years, with a population of around 2,0000, according to the Galápagos Conservation Trust.
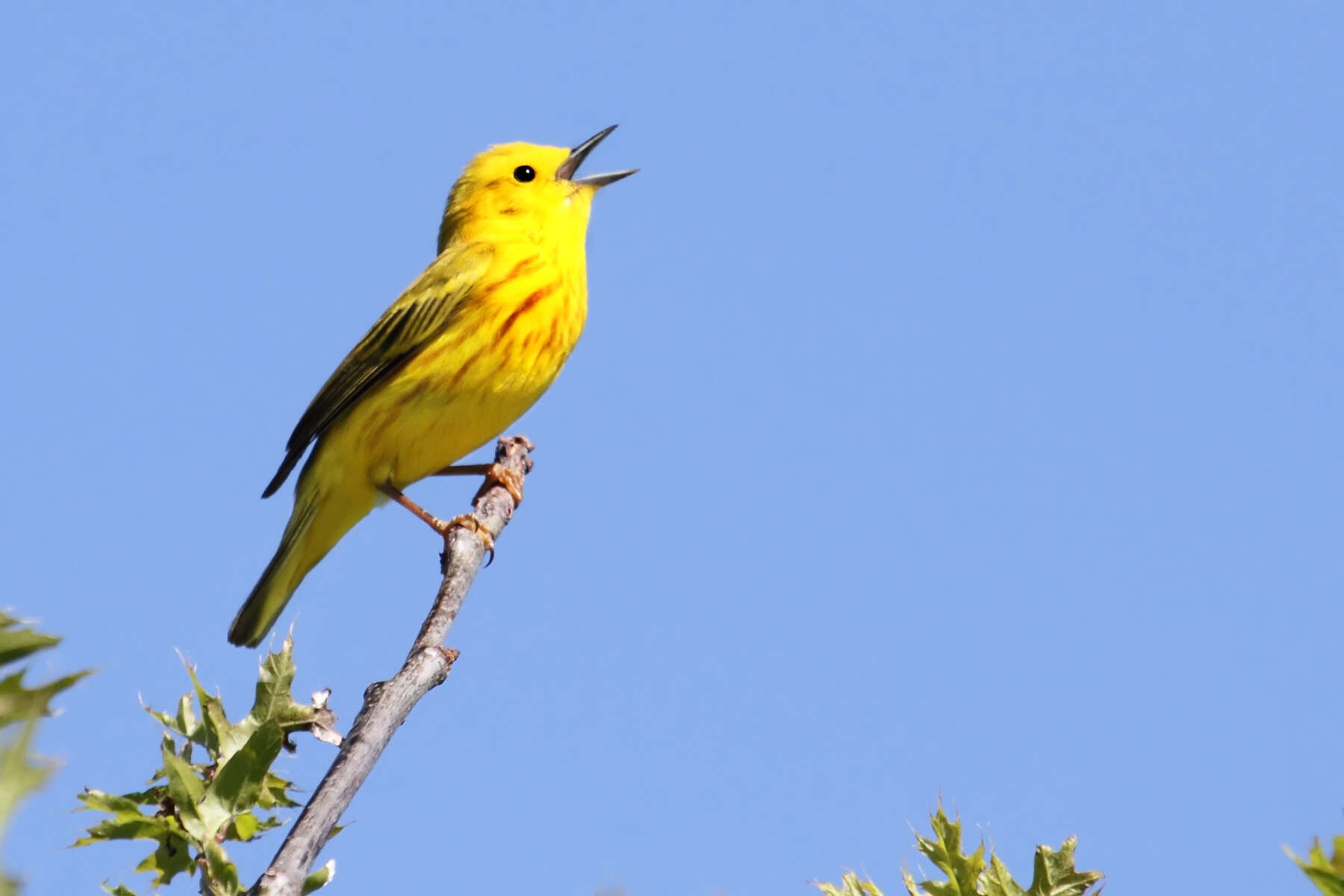
Alarming news came in 2009, when University of Missouri researchers found the malaria parasite in 19 of the 362 Galápagos penguins whose blood they tested, as well as in the small ground finch, the medium ground finch and the yellow warbler. Culex quinquefasciatus, a highly competent mosquito that had already helped introduce Plasmodium to Hawai‘i and New Zealand, was accidentally brought to the Galápagos Islands in 1985. However, blood tests of penguins in 2001 had found no evidence of the malaria parasite. In other words, the mosquito train had been ready and waiting for a while and now its parasite passengers had arrived.
How had Plasmodium reached these islands in the eight years between 2001 and 2009? Suspicion is falling on the bobolink (Dolichonyx oryzivorus), a small New World blackbird and the only passerine songbird that stops over in the Galápagos Islands on its migration from North to South America, after researchers found Plasmodium in 438 bobolink blood samples.
The Galápagos Islands, which have been called a living museum and a showcase of evolution and famously inspired Charles Darwin’s theory of evolution by natural selection, may be on the brink of an ecological disaster similar to Hawai‘i. Although there have not yet been any deaths from avian malaria here, Galápagos penguins, like the yellow-eyed penguins of New Zealand, have evolved for millions of years without encountering the malaria parasite and may have no defenses against it. A single strong El Niño event such as the one in 1982 could decimate their numbers by as much as 77% by warming the water and depleting the fish they feed on and triggering their extinction. Malnutrition could lay the remaining birds open to the malaria parasite.
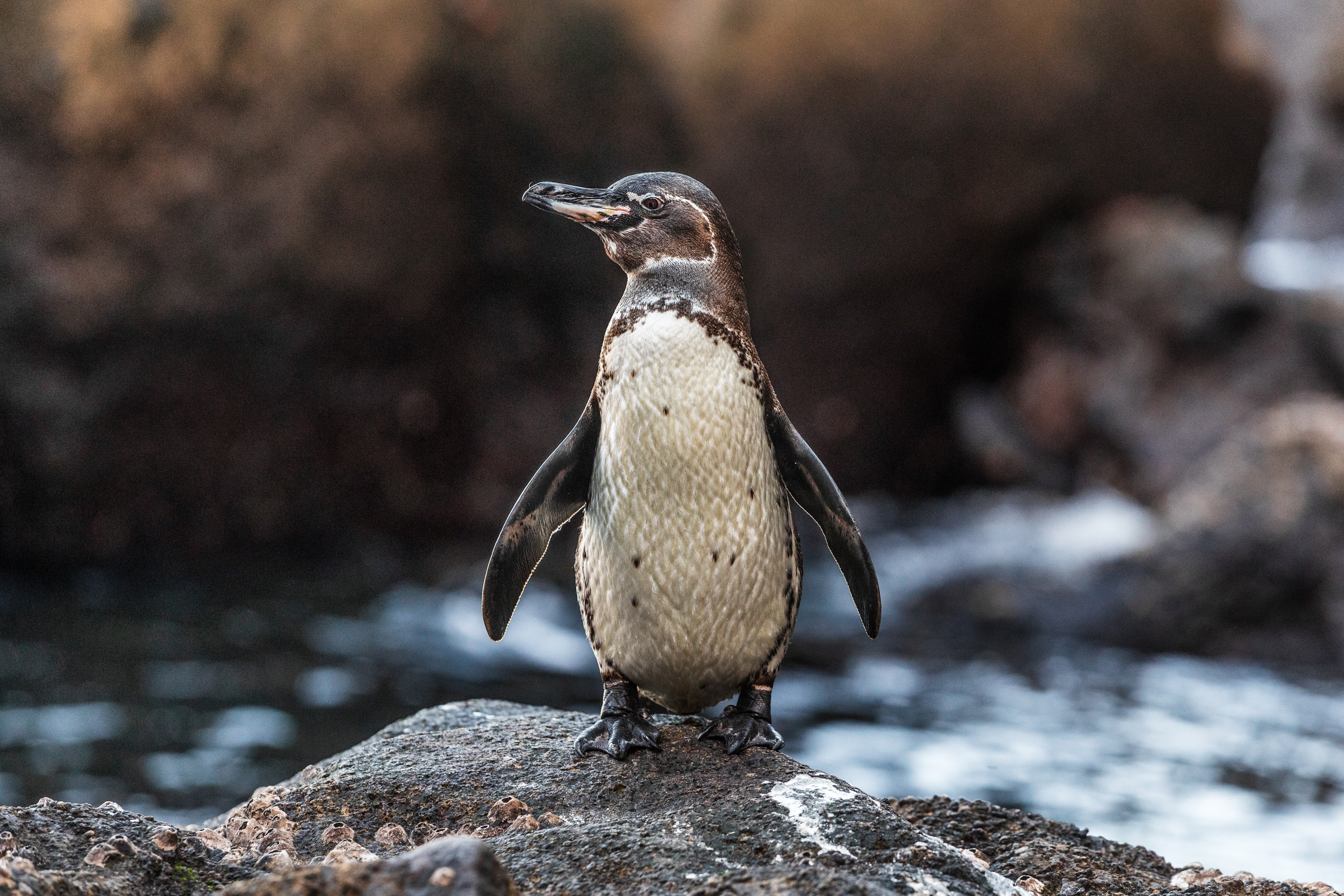
The Future of Birds
Malaria parasites may be among the most intensively investigated vector-borne pathogens in the world, but much remains to be understood about how rapidly changing climate and environments will open up new geographies for Plasmodium, impact its life cycle and affect the dynamics of transmission, infection and mortality. Hawai‘i offers a valuable preview and insights into what might unfold in places like New Zealand, where avian malaria may still be in a ‘sleeper’ stage, and the Galápagos Islands, which may be experiencing the calm before the storm. Some critical research priorities are already clear:
1. Identifying all the mosquito species that are acting as vectors and quantifying their vector competence;
2. Pinpointing the relative roles of climate, land use and habitat disturbance on disease emergence through their influence on the distribution of vectors;
3. Assessing the susceptibility of native birds to infection and identifying naïve species that may be especially susceptible;
4. Better understanding host-community scale dynamics.
The good news is that we may have a head start, thanks partly to the giant leaps of recent decades in understanding human malaria, its mitigation, prevention, and treatment. Some new tools that have been shown to reduce mosquito populations include genetic manipulations, including chemosterilization, engineered transgenes, and the application of bacteria like Wolbachia that live in a symbiotic relationship with the mosquito inside its gut. Though such methods are weapons being tested against avian malaria today, it is always a race against the clock. Mosquitoes and parasites have been at the game for millions of years and have an impressive track record of surviving through adaptive evolution to deal with existential threats.
Sterile Insect Technique
In 1973, the United States Department of Agriculture released around 4.3 million sterile male Anopheles albimanus mosquitoes, using a method known as Sterile Insect Technique (SIT) or chemosterilisation, in El Salvador, where the mean daily production of mosquito pupae was 42,900. The released mosquitoes would compete with wild mosquitoes but produce no larvae when they mated. Over five months, El Salvador saw a 99% reduction in mosquitoes in the project area.
SIT has been tested extensively and with great success on Plasmodium vectors such as Culex quinquefasciatus. SIT’s major weakness is that it can be bypassed by migrating mated females from nearby areas. Such obstacles must be overcome and new techniques such as irradiation explored, and environmental and political concerns addressed before SIT can be deployed widely.
Genetic Engineering
Systems such as CRISPR-Cas can now be used to precisely target and modify specific cargo gene sequences in mosquitoes that reduce the fitness of succeeding generations. The changes can be engineered to be self-limiting so that only female mosquitoes born from a mating between a wild female and a male carrying the cargo gene will die. However, more technological advances are needed to limit unwanted consequences, improve the efficiency of genetic engineering, and develop safety and regulatory measures before this method can be used widely.
Incompatible Insect Technique (IIT)
This method, first used in two Chinese islands to limit dengue-carrying mosquitoes with stunning success, was deployed in Hawaii to control Culex quinquefasciatus, a first with avian malaria mosquito. IIT uses the fact that both mating mosquitoes must be carrying the same species of a naturally occurring bacteria called Wolbachia for the union to produce larvae. By releasing millions of mosquitoes with an incompatible strain of Wolbachia, IIT drastically reduces mosquito populations by ensuring that the resulting eggs are not sterilized and do not hatch.
More research is needed to understand the distribution and diversity of Wolbachia and their dynamics with mosquito hosts to identify mosquito species and Wolbachia strains susceptible to this approach. In species such as Culex pipiens, having a Wolbachia infection of the wPip (Sl) strain could increase receptivity to Plasmodium, which is not good news. More work is needed to develop efficient ways of moving and releasing millions of mosquitoes in remote locations.
Larvicides (Bti, Bs)
Larvicides have not been used here for avian malaria control but with the clock running out and critically endangered bird species now in their hundreds, biological insecticides could offer a solution for the local control of malaria in Hawai‘i. Specifically, two larvicides, Bti (Bacillus thuringiensis israelensis) and Bs (Lisinibacillus (Bacillus) sphaericus), have been tested and proven for the control of mosquito larvae in aquatic environments all over the world.
Bti and Bs occur naturally in soils and have similar modes of action against mosquito larvae. Added to water, the bacteria are ingested by mosquito larvae but once inside, they release a protein crystal, which in turn dissolves to release a toxin that destroys the walls of the mosquito’s midgut. Kaua‘i’s Alaka’i Plateau, the last sanctuary for its endangered honeycreepers, is a perfect proving ground for field-testing these larvicides. In August 2023, trials of Bti and Bs on a 270-acre area in the plateau’s northwest took place. These biorational larvicides are uniquely lethal to mosquito larvae but harmless to humans, birds, fish, animals and plants.
Avian malaria is not the only or even the leading killer of birds in a fast-changing world. They face all the same predicaments as humans when it comes to climate change — toxic air, a warming planet, helter-skelter weather, unpredictable extreme climate events, plastics in the water, garbage everywhere, and so many others. But beyond those, birds are victims of the casual harm humans bring in their wake, from loss of habitat to the introduction of new predators and, time and time again, new diseases.
Rosalie Goldsworthy, who runs the Penguin Rescue Rehabilitation facility in New Zealand, adds an important reminder for all: species do not fall sick, its members do. Without effective tools and treatments, avian malaria will continue taking birds individually. “When a species goes extinct,” she says, “it dies one at a time.”
What she does not add is that the clock is fast running out for Hawaii’s endangered birds. To pull them back from extinction, we may have to save them one species at a time.
Click here for References
History of the discovery of malarial parasites and their vectors
History of the discovery of the malaria parasites and their vectors
USGS article on Avian Malaria
Avian Malaria | U.S. Geological Survey
How Alphonse Laveran discovered the parasite
Laveran and the Discovery of the Malaria Parasite
Excellent CDC video on the malaria cycle with stages
Plasmodia
DNA study of King Tut
Tutankhamen’s Familial DNA Tells Tale of Boy Pharaoh’s Disease and Incest | Scientific American
Everything you should know about avian malaria
What Is Avian Malaria?
Andrea Paez primer on avian malaria
Miranda Paez et al. Parasites & Vectors (2022) 15:208
https://doi.org/10.1186/s13071-022-05327-2
https://www.nasonline.org/publications/biographical-memoirs/memoir-pdfs/maccallum-william.pdf
Brooks, D. R., & Hoberg, E. P. (2008). How will global climate change affect parasite-host assemblages? Trends in Parasitology, 23(11), 571–574.
Handbook for Mosquito Management on National Wildlife Refuges (2018). https://www.fws.gov/sites/default/files/policy/pdfs/MosquitoHandbook_6_2018.pdf
Neuhaus, A. P., Lane, O. P., Kovach, A. I., Conway, M., Kneeland, M. R., & Martinsen, E. S. (2023). Prevalence and diversity of malaria parasites (genus Plasmodium) in the imperiled Saltmarsh Sparrow are greater at northern sites. Ornithological Applications, 125, duad031.
Rogers, D., & Randolph, S. (2000). The global spread of malaria in a future, warmer world. Science, 289(5485), 1763-1766. https://doi.org/10.1126/science.289.5485.1763
Samuel, M. D., Woodworth, B. L., Atkinson, C. T., Hart, P. J., & LaPointe, D. A. (2015). Avian malaria in Hawaiian forest birds: Infection and population impacts across species and elevations. Ecosphere, 6(6), Article 104. https://doi.org/10.1890/ES14-00393.1
Climate Change Assessment: Hawai’i/ Nov 2023
https://nca2023.globalchange.gov/chapter/30/
Everything you should know about avian malaria
What Is Avian Malaria?
Benning, T. L., LaPointe, D., Atkinson, C. T., & Vitousek, P. M. (2002). Interactions of climate change with biological invasions and land use in the Hawaiian Islands: Modeling the fate of endemic birds using a geographic information system. Proceedings of the National Academy of Sciences, 99, 14246-14249.
Ferreira, F. C., Santiago-Alarcón, D., & Braga, É. M. (2020). Diptera vectors of avian Haemosporidians: With emphasis on tropical regions. In D. Santiago-Alarcón & A. Marzal (Eds.), Avian malaria and related parasites in the tropics: Ecology, evolution and systematics (pp. 185–250). Cham, Switzerland: Springer.
Freed, L. A., Cann, R. L., Goff, M. F., Kuntz, W. A., & Bodner, G. R. (2005). Increase in avian malaria at upper elevation in Hawai’i. Condor, 107, 753-764.
Jarvi, S. I., Atkinson, C. T., & Fleischer, R. C. (2001). Immunogenetics and resistance to avian malaria in Hawaiian Honeycreepers (Drepanidinae). Studies in Avian Biology, 22, 254–263.
LaPointe, D. A., Atkinson, C. T., & Jarvi, S. I. (2009). Managing disease. In T. K. Pratt, C. T. Banko, J. D. Jacobi, & B. L. Woodworth (Eds.), Conservation biology of Hawaiian forest birds: Implications for island avifauna (pp. 405-424). Yale University Press.
LaPointe, D. A., T. V. Black, M. Riney, K. W. Brinck, L. H. Crampton, and J. Hite. 2021. Field trials to test new trap technologies for monitoring Culex populations and the efficacy of the biopesticide formulation VectoMax® FG for control of larval Culex quinquefasciatus in the Alaka‘i Plateau, Kaua‘i, Hawaii. Hawai‘i Cooperative Studies Unit Technical Report HCSU-096. University of Hawai‘i at Hilo. 38 pages. https://hilo.hawaii.edu/hcsu/publications
Neddermeyer, J. H., Parise, K. L., Dittmar, E., Kilpatrick, A. M., & Foster, J. T. (2023). Nowhere to fly: Avian malaria is ubiquitous from ocean to summit on a Hawaiian island. Biological Conservation, 279, 109943.
Paxton, E. H., M. Laut, S. Enomoto, and M. Bogardus. 2022. Hawaiian forest bird conservation strategies for minimizing the risk of extinction: Biological and biocultural considerations. Hawai‘i Cooperative Studies Unit Technical Report HCSU-103. University of
Hawai‘i at Hilo, Hawaii, USA. 125 pages. http://hdl.handle.net/10790/5386
Samuel, M. D., Woodworth, B. L., Atkinson, C. T., Hart, P. J., & LaPointe, D. A. (2015). Avian malaria in Hawaiian forest birds: Infection and population impacts across species and elevations. Ecosphere, 6(6), Article 104. https://doi.org/10.1890/ES14-00393.1
Samuel, M. D., Hobbelen, P. H., DeCastro, F., Ahumada, J. A., LaPointe, D. A., Atkinson, C. T., Woodworth, B. L., Hart, P. J., & Duffy, D. C. (2011). The dynamics, transmission, and population impacts of avian malaria in native Hawaiian birds: A modeling approach. Ecological Applications, 21, 2960-2973.
Van Dine, D. L. (1904). Mosquitoes in Hawai’i. Honolulu: Hawaiian Gazette Company.
van Riper, C., & Scott, J. M. (2001). Limiting factors affecting Hawaiian native birds. Studies in Avian Biology, 22, 221–233.
Warner, R. E. (1968). The role of introduced diseases in the extinction of the endemic Hawaiian avifauna. The Condor, 70, 101-120.
The Pathology of Fatal Avian Malaria Due to Plasmodium elongatum (GRW6) and Plasmodium matutinum (LINN1) Infection in New Zealand Kiwi (Apteryx spp.)
https://pdfs.semanticscholar.org/d8e2/d10133956f2fc0e8136c568d6ed80305036f.pdf
D. M. Tompkins & D. M. Gleeson (2006). Relationship between avian malaria distribution and an exotic invasive mosquito in New Zealand, Journal of the Royal Society of New Zealand, 36:2, 51-62, DOI: 10.1080/03014223.2006.9517799
Schoener, E. R., Banda, M., Howe, L., Castro, I. C., & Alley, M. R. (2014). Avian malaria in New Zealand. New Zealand Veterinary Journal. 62(4), 189–198, 2014
When Birds Get Sick. (2020). Kate Evans, New Zealand Geographic, Issue 166, Nov-Dec 2020. https://www.nzgeo.com/stories/when-birds-get-sick/
Loiseau, C., Harrigan, R. J., Cornel, A. J., Guers, S. L., Dodge, M., & Marzec, T. (2012). First evidence and predictions of Plasmodium transmission in Alaskan bird populations. PLoS ONE, 7(9), e44729. https://doi.org/10.1371/journal.pone.0044729
Climate Change Assessment: Alaska/ Nov 2023
Fifth National Climate Assessment 29. Alaska
Avian Malaria in Alaska: The Climate Change Connection
Avian Malaria in Alaska: The Climate Change Connection
Weidhaas, D. E., Breeland, S. G., Lofgren, C. S., Dame, D. A., & Kaiser, R. (1973). Release of chemosterilized males for the control of Anopheles albimanus in El Salvador: Dynamics of the test population. American Journal of Tropical Medicine and Hygiene, 23(2). https://www.ajtmh.org/view/journals/tpmd/23/2/article-p298.xml